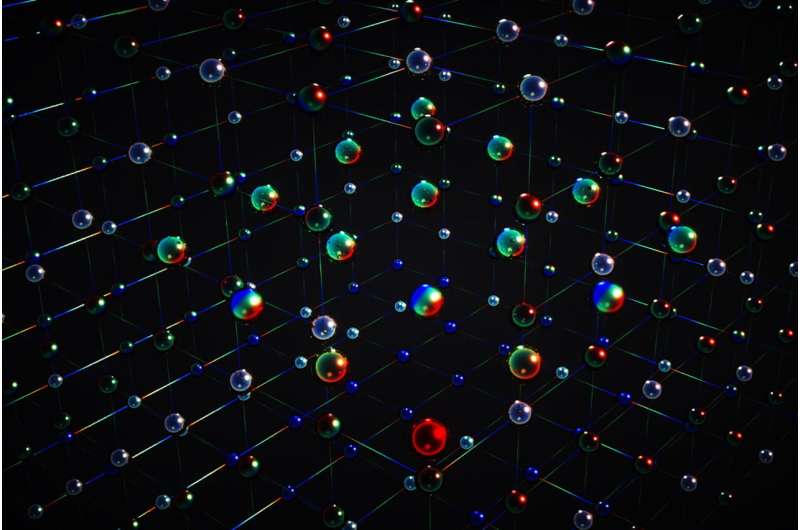
An artist’s visualization of the complex magnetic correlations observed by physicists using a pioneering quantum simulator at Kyoto University that uses ytterbium atoms is about 3 billion times cooler than deep space. The different colors represent the six possible spin states for each atom. The simulator uses up to 300,000 atoms, allowing physicists to directly observe how particles interact in quantum magnets whose complexity is beyond the reach of even the most powerful supercomputers. Credit: Ella Maru Studio/K. Hazard / Rice University
Japanese and American physicists have used atoms about 3 billion times cooler than interstellar space to open a portal into the unexplored realm of quantum magnetism.
Caden Hazzard of Rice University, author of the corresponding theory for a study published today in Nature Physics. “Fermions are not rare particles. They include things like electrons and are one of two types of particles that make up all matter.”
The Kyoto team led by study author Yoshiro Takahashi used a laser to cool fermions, ytterbium atoms, to within one billionth of a degree. absolute zero, the unattainable temperature at which all movement stops. That’s about 3 billion times cooler than interstellar spacewhich is still warm due to the afterglow from the Big Bang.
“The payoff from getting that cool is that the physics is really changing,” Hazzard said. “Physics begins to become quantum mechanical, and it allows you to see new phenomena.”
Atoms obey the laws of quantum dynamics just like electrons and photons, but their quantum behavior becomes apparent only when they are cooled to a fraction of a degree of absolute zero. Physicists have used laser cooling to study the quantum properties of ultracold atoms for more than a quarter of a century. Lasers are used to cool atoms and constrain their motion in 1D, 2D or 3D optical networks or optical channels that can serve as quantum simulators capable of solving complex problems beyond the reach of conventional computers.
Takahashi’s laboratory used optical clamps to simulate the Hubbard model, a commonly used quantum model created in 1963 by theoretical physicist John Hubbard. Physicists use Hubbard’s models to investigate the magnetic behavior of superconducting materials, especially those in which interactions between electrons produce collective behaviour, such as the group interactions of jubilant sports fans performing “wave” in crowded stadiums.
“The thermometer they’re using in Kyoto is one of the important things that our theory provides,” said Hazzard, a professor of physics and astronomy and a member of the Rice Quantum Initiative. “By comparing their measurements with our calculations, we can determine the temperature. The standard temperature is achieved thanks to interesting new physics that have to do with the very high symmetry of the system.”
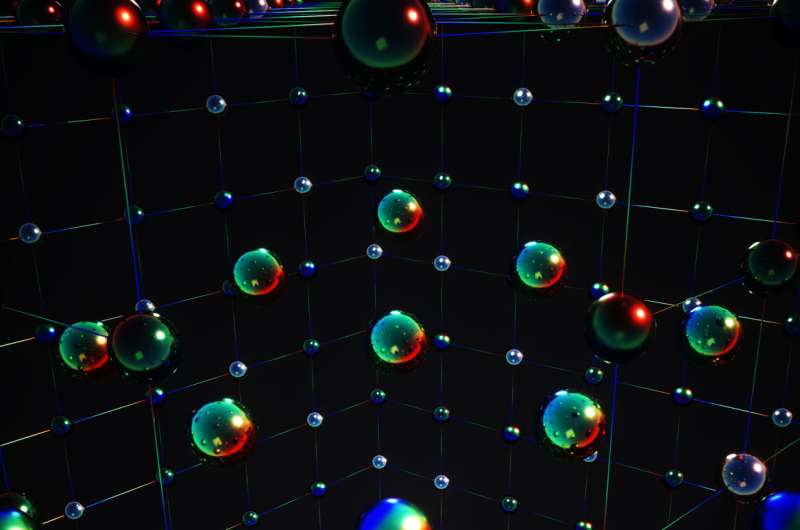
An artist’s visualization of the complex magnetic correlations observed by physicists using a pioneering quantum simulator at Kyoto University that uses ytterbium atoms is about 3 billion times cooler than deep space. The different colors represent the six possible spin states for each atom. The simulator uses up to 300,000 atoms, allowing physicists to directly observe how particles interact in quantum magnets whose complexity is beyond the reach of even the most powerful supercomputers. Credit: Ella Maru Studio/K. Hazard / Rice University
Hubbard’s model simulated in Kyoto has a special symmetry known as SU (N), where SU represents a special unitary group - a mathematical way of describing symmetry - and N indicates the possible spin states of the particles in the model. The higher the value of N, the higher the symmetry of the model and the complexity of the magnetic behaviors it describes. Ytterbium atoms have six possible spin states, and the Kyoto simulation is the first to reveal magnetic correlations in the SU(6) Hubbard model, which is impossible to calculate on a computer.
“That’s the real reason to do this experiment,” Hazard said. “Because we’re eager to learn the physics of this SU(N) Hubbard model.”
Study co-author Eduardo Ibarra-García-Padilla, a graduate student in Hazzard’s research group, said Hubbard’s model aims to capture the minimum components to understand why solids turn into metals, insulators, magnets or superconductors.
“One of the great questions that experiments can explore is the role of symmetry,” Ibarra García Padilla said. “Having the ability to engineer them in the lab is extraordinary. If we can understand that, it could guide us to make real materials with desirable new properties.”
Takahashi’s team has shown that it can trap up to 300,000 atoms in its 3D lattice. Hazard said that an accurate calculation of the behavior of even dozens of particles in the Hubbard SU (6) model is beyond the reach of the most powerful supercomputers. The Kyoto experiments provide physicists with an opportunity to learn how these complex quantum systems work by watching them work.
The results are a major step in that direction, Hazzard said, and include the first observations of particle coordination in the SU(6) Hubbard model.
“Currently, this coordination is short-lived, but as the particles are further cooled, more subtle and exotic phases of matter can emerge,” he said. “One of the interesting things about some of these odd phases is that they are not arranged in a clear pattern, nor are they random. There are correlations, but if you look at two atoms and ask, ‘Are they related?’ “You won’t see them. They’re more accurate. You can’t look at two, three, or even 100 atoms. You have to look at the whole system.”
Physicists do not yet have tools capable of measuring such behavior in the Kyoto experiment. But Hazard said work is already underway to create the tools, and the success of the Kyoto team will catalyze those efforts.
“These systems are very strange and special, but the hope is that by studying and understanding them, we can identify the main components that should be present in real materials,” he said.
Physicists use electrons to make artificial dimensions
Shintaro Taie, Observing antimagnetic correlations in a supercooled SU(N) Hubbard model, Nature Physics (2022). DOI: 10.1038 / s41567-022-01725-6. www.nature.com/articles/s41567-022-01725-6
the quote: SU(N) Material About 3 Billion Times Cooler Than Deep Space (2022, September 1) Retrieved September 1, 2022 from https://phys.org/news/2022-09-sun-billion-colder-deep-space . programming language
This document is subject to copyright. Notwithstanding any fair dealing for the purpose of private study or research, no part may be reproduced without written permission. The content is provided for informational purposes only.